Detecting changes in visual stimuli depends on increases in cortical spiking
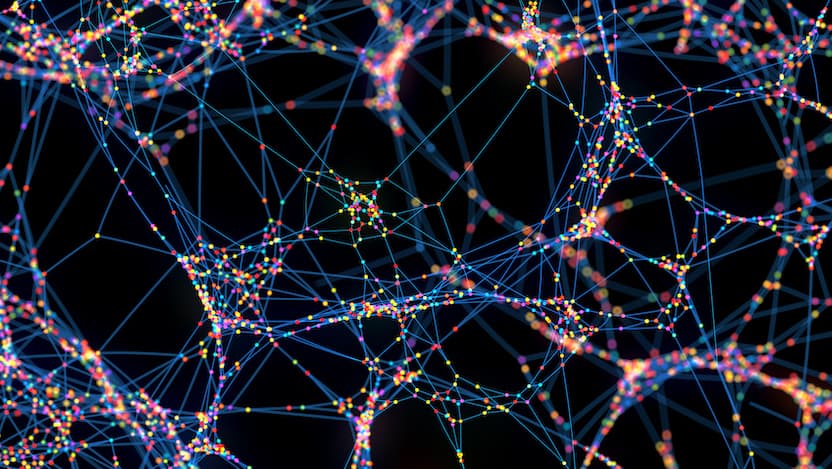
How do our brains translate the signals of millions of neurons into meaningful perceptions of our environment and help guide our behavior? Attempting to answer this question is no small task, but understanding the connection between spiking neurons and our behavior will not only provide insights into the human brain but also will be the key for developing new and innovative neuroprosthetic devices.
A new study published in the Journal of Neuroscience on September 11, 2020 has unlocked one small piece of this puzzle. While studying visual perception in mice, researchers at the University of Chicago found that when neuronal spiking in the primary visual cortex was enhanced using optogenetic stimulation, the mice had an improved ability to detect changes in contrast during a visual perceptual task. Suppressing spiking had the opposite effect. These results provide new understanding into some of the core rules about how the brain processes and interprets information from the activity of neurons.
This project was inspired by past research investigating the role of inhibitory interneurons in the primary visual cortex (V1) during a visual perception task. The researchers used a technique called optogenetics, which uses tiny LEDs and genetically-encoded light-responsive proteins to activate specific groups of neurons in the brain, letting them directly activate the interneurons to determine their effects on behavior.
Stimulation of inhibitory interneurons leads to a reduction in the firing rates of cortical pyramidal neurons, cells that are critical for receiving and transmitting sensory information. In the early stages of cortical visual processing, these neurons code for very basic visual information, such as orientation and color. When stimulating these inhibitory interneurons, the investigators found that the firing rates of pyramidal neurons went down as expected — but were surprised to notice that despite days and even weeks of running the same test, the mice were never able to use reductions in pyramidal cell activity to aid their performance on the visual task.
“This was a really striking observation,” said first author Jackson Cone, PhD, a postdoctoral fellow in the Maunsell Lab at UChicago. “For whatever reason, the mice could not learn to use that inhibitory signal, suggesting that reductions in cortical activity were imperceptible. If we think of the brain like a machine, the optimal machine would use both excitatory and inhibitory signals to understand the environment, coding information in both directions. Our data suggested that when you reduce activity in the cortex, whatever reads out that information downstream can’t detect the change. But why would the brain ignore a signal that could be useful?”
This paradox inspired the team to dig into the phenomenon further, this time by directly modulating the activity of the cortical pyramidal neurons themselves with optogenetics. The researchers stimulated cortical pyramidal neurons while the mice were performing a visual contrast discrimination task and found that the stimulation enabled the mice to perform better on the task.
But because these pyramidal neurons normally have low baseline levels of activity, the team had to get creative to figure out how they could artificially induce a similar decrease in spiking, to produce that inhibitory signal. They developed a new approach, involving a slow increase in optogenetic stimulation to adapt the mice to a new, higher-level of pyramidal cell activity. From there, they could then increase or decrease the stimulation, and determine the changing spike rates’ effects on visual perception.
As they suspected, they saw that suppressing spiking in cortical pyramidal neurons led to worse performance on the visual discrimination task. But why would evolution create a system that on its surface appears to be so inefficient?
The scientists hypothesized that perhaps it had to do with the metabolic limitations of the brain. “We thought that because spikes are energetically expensive, this would put pressure on the brain to minimize the numbers of spikes that it fires,” Cone said. “The consequence would be that the brain has low rates of activity at rest, and because of that, there’s a floor effect; if basal activity is low, you don’t have much of a range for coding information below that baseline.”
Thanks to their location in the multidisciplinary Grossman Institute for Neuroscience, Quantitative Biology and Human Behavior, members of the Maunsell Lab were able to brainstorm a way to answer this question with researchers from the Freedman Lab. Together, the team developed an artificial neural network to perform the same task as the mice they had been studying. Then, they imposed metabolic restraints to the artificial network that would penalize the system every time it fired a spike, similar to the energy expenditure seen with real neuronal spikes.
“As we increased the penalty for a spike, we saw less basal activity in the model, and the more likely it was to simply ignore reductions in firing,” Cone said. “The model saw that there was very limited information in those signals in the first place, so it essentially started ignoring them, just like what we saw in the mice.”
These experiments showed that the cerebral cortex seems to control behaviors exclusively with increases in brain cell activity due to those energetic restraints.
“When we increased the activity of cells in V1, the mice were easily able to detect and use that change, but when we decreased brain cell activity by even bigger amounts the animals were completely unable to detect the change,” said senior author John Maunsell, PhD, the Albert D. Lasker Professor of Neurobiology and Director of the Grossman Institute. “This points to the brain being wired to use increases in activity exclusively. It’s a clever approach because most of the time none of the cells are very active, which can save a lot of energy. Brains take a tremendous amount of energy, about 20 percent of all the calories we eat, so this efficiency might be critical.”
These results will help guide future research on how our brains code and translate visual information. “These data have let us learn a lot more about exactly what information the brain cares about when it comes to the processing of neuronal signals,” said co-author Morgan Bade, a research specialist and lab manager in the Maunsell Lab. “Really understanding how these signals are used in the most fundamental sense will be important for all kinds of biomedical research going forward, particularly when it comes to brain-machine interfaces and neuroprosthetic devices.”
In the future, the team plans to dig further into this phenomenon, using new techniques including two-photon microscopy to control the activity of more refined groups of cells and to tease apart the response to those signals. “Natural visual stimuli lead to very complex patterns of activity in the brain, and the optogenetic stimulations we used in this study are crude by comparison,” Cone said. “Ultimately, our goal is to be able to read out neuronal activity and replicate real-time brain signals artificially to ask precise questions about how the brain translates the activity of neurons into perception and action.”
The study, “Mice preferentially use increases in cerebral cortex spiking to detect changes in visual stimuli,” was supported by the NIH (U01-NS090576 and NIH U19-NS107464), an Albert O. Beckman Postdoctoral Fellowship, and the Vannevar Bush Faculty Fellowship. Additional authors include Nicolas Masse, Elizabeth Page, and David Freedman of the University of Chicago.